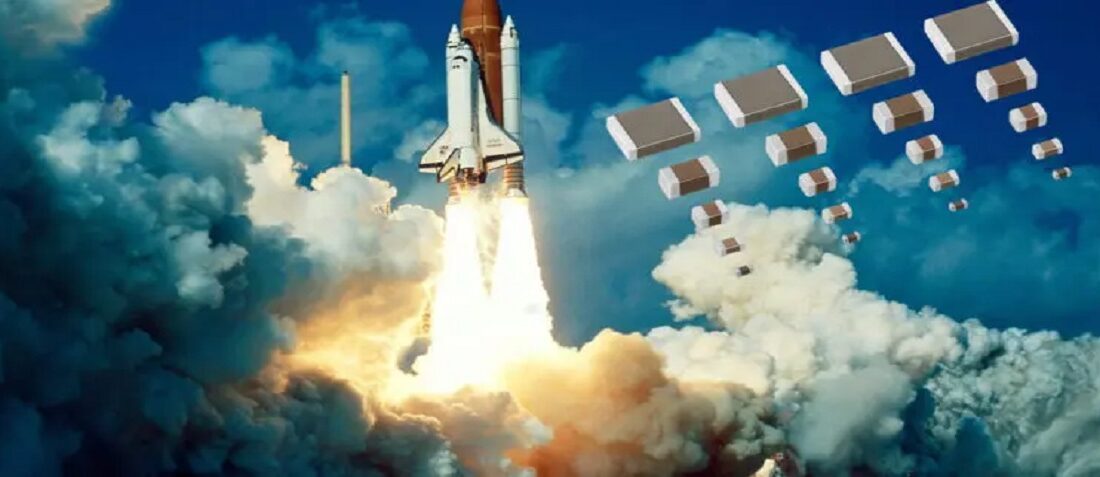
Passives Components Selection Guide for Space-Grade Switching Regulators
- Posted by doEEEt Media Group
- On February 3, 2021
- 0
With the increasing use of low-voltage, high-current loads such as FPGAs within spacecraft avionics, engineers increasingly have to design-in switching-mode regulators to generate supply rails. Selection of passive electronic components is one of the key items for reliable operation. Dr Rajan Bedi, CEO and founder of Spaceships, discuss its selection guide in an article published by EDN.
Most of the qualified parts have integrated the switching and low-side FETs, but require an external inductor and input and output capacitors. These passives’ choice is significant as they determine the conversion quality, i.e. input and output ripple, load regulation, and response to single-event effects (SEEs).
Power supply design is by its very nature full of trade-offs and compromises such as cost, size, performance, and reliability. However, there is also scope to optimise and reduce the dimensions and mass of the energy-storing passives.
Switching DC-DCs regulate the output voltage based on energy flow into and from the DC-DC by controlling the on and off states’ duty cycle. When the switch is conducting, energy flows from the input source into the converter and in the case of a buck, some of this gets stored in the inductor as magnetic energy (0.5*LI2). In contrast, some get delivered directly to the output (forward topology). Similarly, during the off interval, energy is delivered from the converter to the load. For a buck, this comes from the inductor, where it was previously stored when the FET was on.
The energy added to the inductor during the on-time is always equal to that delivered to the load when the switch is off, i.e. the inductor ends each cycle with the same current and energy it started with; the definition of steady-state!
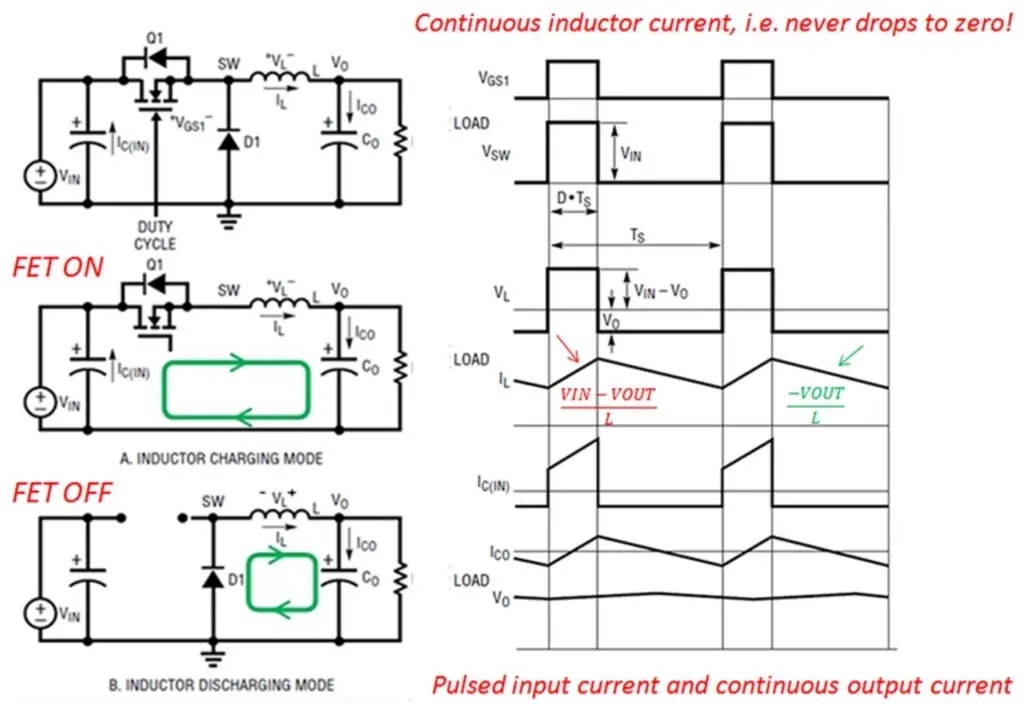
Figure 1 This diagram shows the switching cycle of a buck regulator.
As an example, assuming a switching frequency of 500 kHz, an input of +5.5V, a load voltage of +0.95V, a load current of 18 A, and a conversion efficiency of 90%, the resulting input and output powers are 19 and 17.1 W respectively. The source energy drawn during each switching cycle is 38 µJ, and the energy output when the FET is off is 34.2 µJ. At a frequency of 500 kHz, the total energy processed by the regulator is 17.1 J/s, or 17.1 W.
When we add energy to an inductor, the current ramps up linearly. When we remove the energy, the current ramps down, resulting in an observed ac current ripple. Analogously, when we add energy to a capacitor, the voltage across it ramps up linearly. When we remove the energy, voltage ramps down, resulting in an observed ac voltage ripple. The switching action continuously adds and removes energy, producing a current ripple through the inductor and a voltage across capacitors. For both, there are guidelines for the maximum amount of tolerable ac variation relative to its dc level.
For a buck regulator, the inductor stores the input energy in its magnetic field when the switching FET is on and delivers this to the load when the high-side transistor is off.
For example, to generate a +0.95V rail at 18 A, the following table lists the absolute peak-to-peak inductor current ripple in amps and as a percentage of Iload, as well as the resulting inductance as a function of r:
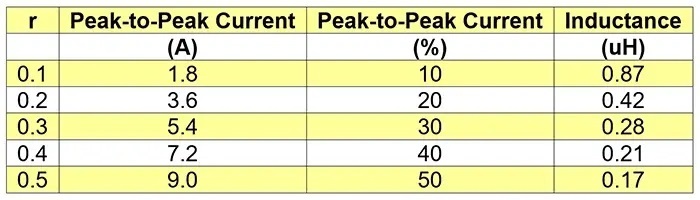
Table 1 Calculation of inductor ripple and inductance
The value of the inductance is inversely proportional to load current and switching frequency while its physical size and mass are proportional to Iload.
A fully-integrated, space-grade buck regulator offers an overall smaller form factor and is easier to design-in while having to add an external magnetic and passives gives the designer more control over the level of current ripple, and hence the voltage variation passed to the load.
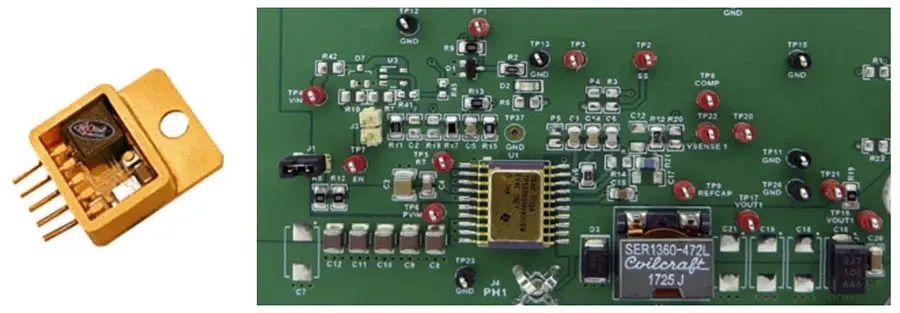
Figure 2 Compare fully-integrated vs. non-integrated space-grade switching regulators.
A large inductance produces less current ripple, resulting in lower voltage fluctuation in the load. A larger inductance, because of its natural tendency to oppose a change in current, slows the regulator’s transient response and increases power loss due to a bigger intrinsic dc resistance. A smaller inductance improves the regulation speed but increases the amount of output ripple and risks the inductor being driven into saturation. It is essential that the inductors’ RMS current and saturation current ratings are not exceeded, with the latter more significant than the calculated peak value. The physical size of the magnet must match its energy-handling capability and a bigger core is required to handle higher powers. Please also check the inductance tolerance, which can vary by up to 30% for some suppliers, resulting in a higher than desired ripple current.
The inductor current ripple appears as a variation in the output voltage, and one of the functions of the output capacitance is to reduce the amount of ripple seen by the load. Capacitor ESR and ESL are important parameters determining the output voltage ripple associated with the inductor current.
A capacitor naturally resists changes in ac. voltage, and once it is charged has a tendency to maintain the voltage, especially if there is a ripple riding on top of the dc. The capacitor, if appropriately sized, will be too slow to keep up with voltage fluctuations due to ripple, so they get filtered out.
When the FET is off, the input is disconnected from the output. However, the load will always require a continuous flow of energy. During this time, the output capacitance must also store sufficient charge to provide the load with an output: when the switch is off, the inductor current decreases while supplying the load, and the output capacitance buffers this current variation so the load sees a nearly constant voltage.
The output capacitance also determines how the regulator responds to a large change in load current. It must be sized appropriately to supply the load while the DC-DC cannot and until the latter’s feedback control loop can respond.
The LC filter formed by the inductor and output capacitance removes the ac component of the switching waveform to output an average (dc) voltage. For a buck regulator, the output capacitance is repeatedly charged and discharged by the ripple centered on the load voltage. Trace Ico in Figure 1 plots the continuous current flowing into the output capacitance.
Typically, various different capacitors are used in parallel to minimize the equivalent series resistance (ESR) and equivalent series inductance (ESL) contributions to output ripple and current sharing to ensure reliable operation. Parts are chosen based on their rated ripple current, working voltage, and parasitics.
Figure 3 plots the ripple-voltage contributions generated by ESR, ESL, and capacitance. The ESR contribution is resistance * current, the capacitance component is the integration of current and time, producing triangular waves, and the ESL component can be expressed as a derivative, with an instantaneous spike occurring at each switching instant producing high-frequency, pulse-like noise.
The peak-to-peak voltage ripple at the output is inversely proportional to capacitance and switching frequency but proportional to load current, ESR, and ESL.
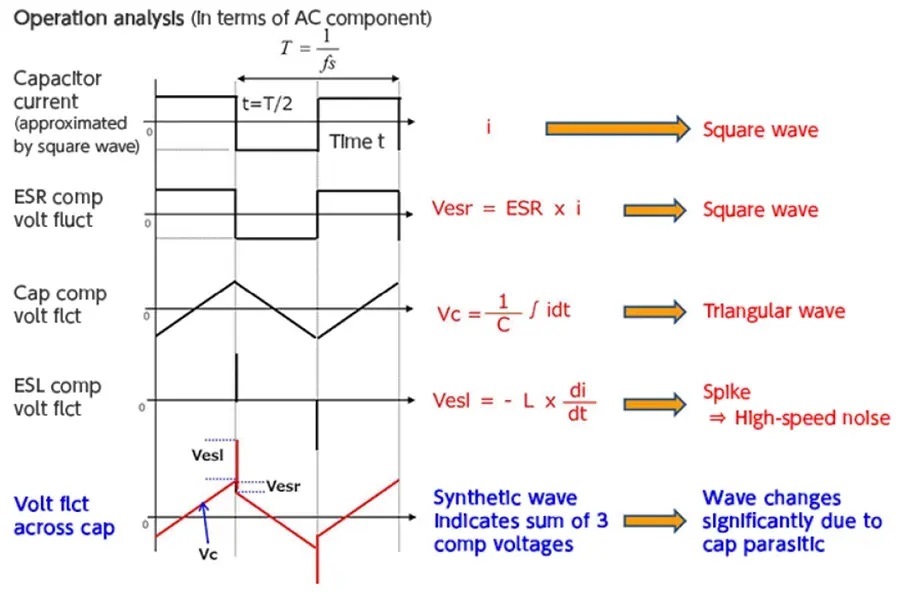
Figure 3 This operation analysis shows the impact of ESR, ESL, and capacitance on ripple voltage.
Figure 4 compares the ripple voltage measured from tantalum and multi-layer ceramic capacitors (MLCCs). MLCCs have lower intrinsic ESR and increasing the amount of capacitance reduces ripple further.
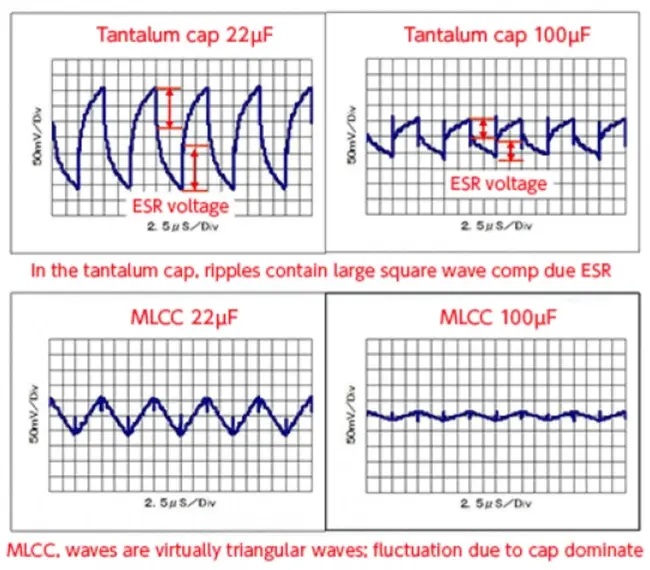
Figure 4 These graphs show the impact of capacitor type and capacitance on ripple voltage.
Input capacitance reduces the source ripple voltage seen at the switch of the DC-DC to a level that can be handled by the bulk capacitors without impacting the latter’s ESR dissipation. For a buck regulator, the ac part of its input current is supplied by the capacitance when the FET is on and conducting. When the high-side switch is off, the input capacitance recharges, and trace Ic(IN) in Figure 1 plots its discontinuous, trapezoidal waveform with high di/dt and peak-to-peak amplitude. The source is not able to generate such a rapidly-changing pulsating current.
Bulk input capacitance minimizes source voltage deviations to ensure a stable output during load transients. The higher the capacitance, the lower the perturbation, which is directly proportional to changes in load current.
A good starting place to determine the amount of input capacitance is to specify the target level of peak-to-peak voltage ripple, which is inversely proportional to capacitance and switching frequency, but proportional to load current and ESR.
For a buck regulator, the input capacitor’s RMS current is much higher than that in the output capacitor, and the former is determined by its stress requirements, whereas on the output, it is simply the maximum allowed load ripple that determines the amount of capacitance. The largest permissible RMS current for a capacitor can be calculated from its maximum power consumption derived from its case size, ESR, and tolerable temperature rise.
Typically, various capacitors are used in parallel to minimize the ESR and ESL contributions to the input ripple and current sharing to ensure reliable operation. Parts are chosen based on their rated ripple current, working voltage, self-heating, ESR, and ESL, with multi-layer ceramics used because of their low parasitics. The observed ripple, therefore, is almost entirely due to the actual capacitance, and attention must be paid to thermal and dc bias effects on the value of capacitors. Dielectrics, which are less sensitive to temperature, are typically used to minimize changes in capacitance. The higher the temperature, the lower the reliability, including lifetime.
There are many trade-offs when designing a buck regulator: a higher switching frequency reduces the level of ripple voltage in the input and output capacitors as well as decreases the amount of capacitance required by the DC-DC. However, reducing the latter increases the amplitude of the voltage ripple, and switching at a faster rate increases ac losses, impacting efficiency. There are also opportunities for optimization, e.g., for a given voltage ripple target; the capacitance can be decreased provided the switching frequency is increased to avail of smaller energy-storage components.
A smaller inductance has lower DCR, improves transient response, and less output capacitance is required for a given transient performance. A more considerable inductance results in a lower ripple current hence less capacitance is required for an equivalent output ripple.
If the inductor’s saturation current is exceeded, its inductance will drop, increasing the ripple current: a 30% drop in inductance will increase the ripple current by > 40% and saturate the core. Shielded inductors reduce EMI and potential interference but are slightly more expensive because of increased manufacturing costs. Shielding tends to lower the saturation current, lowering the maximum allowable RMS current. Lsat also varies with temperature!
Source: EDN
- Space-Grade components available for immediate delivery - April 10, 2025
- Exclusive stock on doEEEt: How to access and request - April 10, 2025
- Managing EEE components for LEO and lower cost space missions - December 17, 2024
0 comments on Passives Components Selection Guide for Space-Grade Switching Regulators